Telomerase Insufficiency and Genome Integrity
- Sanaa Kirpalani
- Nov 30, 2024
- 19 min read
Abstract
Telomere replication is the process of adding repetitive sequences of nucleic base pairs onto the ends of chromosomal DNA in order to lengthen the life and health of the cell. Many theories about the origin of telomerase in eukaryotic cells, why the telomerase becomes dormant, and the development of telomerase reverse transcriptase (TERT) in eukaryotes have been proposed over the past few decades to fill the gap in research telomerase insufficiency and its impacts on genome integrity. This review discusses the history of telomeres and understands the various ongoing theories speculating the introduction of telomerase in eukaryotes and telomerase insufficiency resulting in differing circumstances for the cell. Furthermore, the relevance of theories such as the Hayflick limit on their corresponding results and how it can lead to cellular senescence.
Keywords: Telomerase insufficiency, telomere, Telomerase reverse transcriptase (TERT), telomere replication, telomerase, telomerase origin, cellular senescence, Hayflick limit
Introduction
Telomeres are the ends of the chromosomes that consist of tandem repeats of nucleic base pairs that serve as a protective cap for chromatin and prevent DNA from becoming tangled, rendering them irreplicable (1, 2). During the process of meiosis and mitosis, the double-helix unwinds and one out of the two strands in the double-helix becomes a template strand. DNA replication will then occur, and the leading strand will completely replicate. Unfortunately, the lagging strand will be one telomere repeat short due to the absence of an RNA primer to initiate a final repeat at the 3’ end (3). As a result, an overhang of the 5’ end will occur and create complications later on for the function of the telomere. Telomerase enzymes are the ribonucleoprotein (RNP) polymerase responsible for adding the guanine-rich sequences- using the telomere RNA- onto the telomere through telomerase reverse transcriptase (TERT)- a replicating capability of the enzyme through copying the single-stranded RNA blueprint and creating a double-stranded DNA segment that can be integrated into the genome and other reverse transcriptase (RT) mechanisms (4, 5, 6). However, as we get older, the telomerase activity decreases, resulting in the telomere replication problem, the idea that when the telomere approaches an impediment in the replication process which will produce a stress response that alters the telomere length homeostasis (7, 8). As a cell increasingly divides and the effectiveness of the telomerase enzyme gradually decreases, complications begin to arise due to the genome instability that this inverse relationship generates.
Understanding the functions and roles of the telomeres and telomerase enzymes in eventual death evokes many unanswered controversial questions concerning immortality, cancer, and every age-related disease known to man (9). Exploring potential answers to these questions while gaining a new perspective on the biological function of telomeres may allow more research and progression in this topic of discussion. In order to completely grasp the functionality of the enzymes and telomeres in this review, the origin, structure, risks, and studies of telomeres and the various enzymes involved must be discussed in depth before analyzing the gravity of their capabilities.
Origin of Telomerase Enzymes
The concept of a telomere originated in the late 1930’s when Herman Muller observed peculiar repetitive nucleic base patterns on eukaryotic chromosomal ends during a study on Drosophila melanogaster and later studied by Barbara McClintock in Zea mays (10). Amazingly enough, these discoveries preceded the understanding that DNA was known to be the genetic material and formed a Watson-Crick double helix structure in 1953, showing just how ahead of their time these discoveries were (10). Their groundbreaking observations of the non-coding DNA segments led to a series of further studies directed towards understanding their purpose and importance in eukaryotic organisms. Following these discoveries would come a Nobel prize worthy observation in 1984 from Elizabeth Blackburn and Carol Greider of the telomerase enzyme (11). Their studies not only allowed them to discover the enzyme, but also to understand much more of the effects they have on meiosis, mitosis, and overall age of the cell. These individual discoveries each contributed greatly to the comprehension of our biological functions, and each acted as a puzzle piece in the quest to find cures for cancer and many age-related diseases.
In a collaborated study between Elizabeth Blackburn and Jack Szostak in 1981, it was understood that telomere function could be preserved from organism to organism, more specifically from Tetrahymena to Saccharomyces cerevisiae (12). This observation opened the idea to many other possibilities with telomeres in eukaryotic chromosomes as well as inspired the question, how did telomerase enzymes appear in humans? Although there is no definite answer to this, there are some possible solutions as to how it may have come about. The first being that the presence of the TERT protein in early eukaryotes suggests that telomerase originated as an early RT that created an RNA template which later evolved into a telomerase ribonucleoprotein (13). This idea shows that, although telomerase is commonly found in eukaryotic, bacterial, and fungal lineages, the presence of the RT factor in eukaryotes is what may have allowed for the telomerase enzyme to appear more commonly in humans. Another perspective observes the T-loop structures found at the ends of telomeres that serve as protective caps (14, 15). It was thought that the T-loop structure invaded the telomere structure to protect the chromosomal ends from DNA repairment, ultimately allowing the telomeres to employ a recombination-dependent replication (RDR) technique; commonly used on homologous chromosomes. The result being that the RDR enzymes would approach the ends of the chromosome and form the T-loop structure, allowing the telomeres to replicate through the RDR process. Another possibility is that the development of telomerase in humans may have resulted from a retrotransposon that lost the place where the DNA was formerly integrated and became linked to a new endonuclease domain of non-coding DNA, more generally known as the telomere (13). This solution provides an understanding of how an ancient retrotransposon, commonly observed in fungal ancestry, developed into a fully functional telomerase enzyme. Furthermore, the fungal lineage aspect of this explanation justifies the complex depth of the structure of telomere RNA (TR). For example, complex primitive pseudoknots and D-loops found in the structure of the TR are most likely a result of their common appearance in protozoa and other fungal organisms (13, 14, 16).
Although little is known about the biological makeup of TR, more theories are surfacing as to how telomerase enzymes appeared in humans and how their alleged diverse lineages affect the functions that they perform every day. Different futuristic perspectives may provide an explanation for the many unanswered questions that linger in complex structures of telomerases and help create a more accurate origin story for these amazing RNP enzymes.
Structure and Functionality of Telomerase and Telomeres
The early discovery of non-coding transcription at telomeres showed the world just how complex the structure and function of TR and telomerase were (12). Understanding the biological makeup of these complex structures and how they combine in unison to carry out functions, such as TERT or retrotransposon, is crucial in understanding why they face many replicative problems.
Because the telomerase is derived from a normal ribosome, it consists of the two similar subunits: TR and the TERT protein (15).
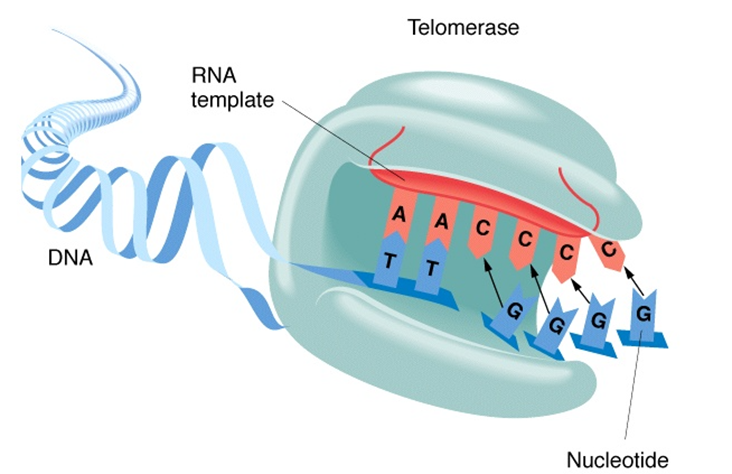
Figure 1
Telomere Elongation Mechanism
When the telomerase is active, the TR (the red RNA in figure 1) and the TERT protein (the light blue structure in figure 1) will assemble and initiate translation to repair the telomere from damage experienced during the cell cycle (17). Like normal translation, the complementary nucleotides will match their TR nucleotides adjacent and attach to the strand of DNA.
The TERT protein assembles with the RNA of the telomere and creates an exact opposite of the RNA template to form a single strand of DNA (the light blue strand shown in figure 1). This single-stranded DNA then copies itself and forms a double helix of the tandem repeats in the telomere. This DNA is now ready to be implemented into the telomere and proliferate the cell (14, 18). This process is reliant on many DNA regulating proteins and enzymes which facilitate and ensure the process of replication is correct. Additionally, there are 6 telomere specific proteins that play roles in the protection, regulation, and elongation of telomeres: TRF1 (telomere repeat binding factor 1), TRF2 (telomere repeat binding factor 2), hRap (repressor/activator protein), POT1 (protection of telomeres 1), and TPP (a POT1 interacting protein) (19, 15). Some of these proteins are present during cell division and carry out functions such as telomere sequence binding facilitators, initiators of T-loop structures, protecting telomeric termini from nucleases, checkpoint locations, regulating telomere length in specific cells, mediators between other proteins, or even repressor/ activator proteins. All these proteins and enzymes are essential in the elongation of telomeres and the activity of telomerase.
Although the science community is not fully aware of all the functions that telomeres perform, it is known that they are often underrated and commonly reduced to their simple reputation: Repeats of unimportant DNA. On the contrary, they are the main reason that the cell cycle is functional. Their unique ability to attach to the spindle fibers and anchor the chromosomes to the inner nuclear membrane assures that they are held in place during metaphase and anaphase. Due to the polarity of the nuclear membrane, the telomeres cluster together and facilitate pairings of homologous chromosomes to ensure each daughter cell receives the right type and amount of DNA (20). Another vital function of telomeres is to shield the natural chromosomal termini from DNA repair mechanisms as well as help to differentiate any possible intrachromosomal double helix breaks that may occur (21). The structure of the telomeres is an important aspect of cell division. For example, the intrachromosomal DNA should be easily accessible for cellular division to avoid a complex and time-consuming process when cell division is needed in a short amount of time, i.e. recovering from an injury (19). Telomeres and telomerase are also capable of simultaneously positioning themselves in different ways after they have undergone telomeric repeat synthesis (22). They can either both re-position themselves for another processive repeat synthesis and revert to creating single-stranded DNA repeats or the telomerase can dissociate from the telomere (22, 23). Telomerase flexibility to bind to the telomere and create a site of replication or detach completely from each other is a tremendously important function of the telomere and enable it to efficiently carry out many different functions all while maintaining genome stability.
Each of the functions of telomeres aforementioned, are significant for any organism to grow, especially when repairing cells and tissues from injury or replacing older cells on the verge of apoptosis. Their ability to precisely guide the genetic material to their correct locations and provide a replication site for telomerase to attach, all in a rapid and proactive manner is not something that should be overlooked nor underappreciated. It is quite literally the reason we can grow as individuals, grow as a population, reproduce, and maintain our health. Telomeres are the reason organisms function the way they do.
Theories for Telomerase Insufficiency
The study surrounding the activation of telomerase has been a field of interest for many scientists for a while. The questions of “what regulates their activity” or “why are they not as active towards the middle and end of human life” have left holes in medicine that are being investigated daily. Although there is not one singular correct answer as to what causes this in humans, there are several theories that have arisen throughout the years providing a possible solution as to why our telomerase enzymes go from extremely active during childhood to barely noticeable in adulthood.
It is believed that the telomerases ability to access the telomere is regulated through different stages of the cell cycle (19). For instance, during the synthesis phase of the cell cycle/interphase, the telomerase elongates the DNA, causing it to be more active and present during this segment of the whole process. It is also believed that the cell cycle elongates some of the shortest telomeres in hopes of increasing the area at which the telomerase can attach to the telomere. However, due to the inability of telomeres to be completely replicated after every cell division, the telomerase has decreased area to attach to the telomere, resulting in decreased activity. This theory also depends on the number and formation of telomeric proteins. A greater ratio of TRF1 proteins to telomeric length could lead to stronger connections and a heavily compacted telomere structure, which would consequently decrease the area where the telomerase enzyme can bind to the telomere. Because TRF1 proteins ensure correct telomere replication, it is understandable that as we age, more TRF1 proteins will attach to the unstable telomere and block any room for the telomerase enzyme to actually replicate (6). Another theory suggests that because telomerase functions as an RNP enzyme, they require stable interactions between the TERT proteins and TR (13). Because the TR template provides a weaker connection with TERT, a second and stronger TERT binding site located near the original interaction would create a 3-way junction and stabilize the interaction. However, because there would not always be a second TERT with a strong binding site to rely on, some interactions are left weak. This is a problem for the telomerase because the telomere replication process is functional only when the telomerase can quickly bind to the telomere and carry out its function without interruptions (24). It must not interact with the other proteins on the telomerase, which becomes very hard when the interactions between the TERT and TR are weak and have high affinity for more interactions. According to a related notion, the complex called Shelterin, which is made up of six proteins exclusive to telomeres, is involved in the deactivation of telomerase enzymes (25, 26). They function as recruiters of TERT proteins to elongate the 3’ overhang of the lagging strand (7). This TERT subunit is inhibited by a CTC1-STN1-TEN1 complex (CST), which consequently acts as a telomerase inhibitor. These similar theories suggest that the telomerase is abundant and functional, but the presence of external stimuli is essentially inhibiting the telomerase from initiating reverse transcriptase.
Some less researched theories include that the number of telomerase enzymes is insufficient to copy each telomere in the human body, every time a cell divides (27). This could be a possibility if the gene that codes for the telomerase enzyme is mutated or silenced via epigenetics, but this is a theory that will apply only to individuals rather than an entire population. Similarly, studies researching the timing of the telomeric sequence replication have questioned if the time allotted for replication is insufficient to thoroughly copy the telomere (7). This is unlikely as well because closer looks at the replication of the telomere clearly show that the leading strand has been completely copied but the lagging strand has an overhang due to the lack of RNA primer at the 3’ end, which does not explain how this phenomenon impacts a whole population (3).
Problems Resulting from Telomerase Insufficiency
The problems that arise with telomerase insufficiencies and the telomere replication problem generally show up at the end of an individual's lifespan. As humans age, various signals indicating the telomere length is decreasing rapidly start to appear. Whether in the form of wrinkles or cancer, it essentially means the same thing in terms of telomeric length. Some hallmarks can be overlooked while others include increased susceptibility to tumor formation, sacrificing genome integrity, and end-to-end fusion; all of which can lead to age-related diseases and death.
When a human experiences the side effects that come with aging, there is one main underlying principle that explains every aspect of why that happens; the Hayflick limit. Leonard Hayflick demonstrated that there is a threshold limit as to the number of times a cell could divide before it begins to age and eventually cause cellular senescence (2, 13, 28). He showed this by observing cell cultures go through the cycles of mitosis and watched as they slowly deteriorate. Furthermore, without the telomerase to repair the lost repeat, the telomere would shorten (3, 9).
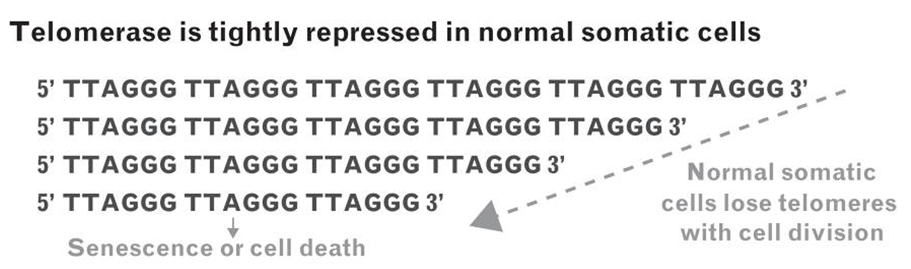
Figure 2
Telomeres entering programmed cellular senescence
Telomerase is inactive in somatic cells, thus when a cell loses a telomeric repeat after each cell division, it is not repaired and continues to shorten (9). Eventually the telomere will become too short and unstable, causing the cell to initiate cellular senescence.
His limit states that all cell types could only divide 40-60 times before entering a programmed cell death, explaining all of the underlying effects of old age and why it is crucial for telomerase activity to be abundant (2). As a cell approaches the Hayflick limit and the telomere continues to shorten, the cell undergoes mitochondrial dysfunction (as portrayed in figure 2) (29). This is a major result of telomerase insufficiency because as the mitochondrial functions start to decline, the cell's source of energy (ATP) decreases and the cell’s ability to carry out necessary functions can lead to reduced body weight, reduced bone mineral density, premature aging phenotypes, etc. All these impacts can rapidly snowball and severely impact the health of the cells and the individual as a whole. Another result of telomerase insufficiency occurs with end-to-end fusion and its impacts on genome integrity (30). End-to-end fusion forms when the telomere is too short and the intrachromosomal DNA is exposed and processed as if it were a DNA double strand break (31). Because of this, it is then processed by unwanted DNA damage repair proteins which will fuse the ends of the double-helix together and eventually lead to accidental DNA recombination and genomic duplications (32). Altering the genome sequence will lead to genome instability and erratic changes in the DNA, which can cause an unknown number of deadly outcomes among the more commonly known ones such as age-related diseases and cancer.
One of the deadly outcomes of accidental DNA recombination is the activation of oncogenic activity in a cell. As the telomeres are shortened, there is a possibility that if the DNA damage repair checkpoint is bypassed, they could become cancerous (33). Another possible way for cancer to develop from genome instability is because end-to-end fusion can create mutations from the erratic changes of the genome, mutants located on the caretaker genes whose main function is to maintain genome stability, may initiate a cancerous response (34). Finally, the most common induction of cancer in a cell occurs if a cell is experiencing abnormally short telomeres, sometimes telomerase activity will greatly increase to avoid crisis and temporarily save the cell from apoptosis and restore telomere stabilization (9).

Figure 3
Cells pathway to oncogenic potential
Oncogene expression causes uncontrolled progression through the cell cycle, giving it tumor potential (26). Further telomere shortening gives rise to senescence, loss of cells ability to divide. p53 and p16 proteins are responsible for cell cycle regulation, so a decrease of these proteins causes cell crisis. To preserve the life of the cell, it will reactivate the once dormant telomerase via stress. Once again, allowing the cell the potential to become cancerous.
However, if the telomerase activity continues to reactivate, the cell is most likely to exhibit oncogenesis because it has been given an opportunity to divide an infinite number of times. Thus, cancer is mostly detected in cells with short, stable telomeres. Additionally, as shown in figure 3, the correlation between shortening of telomeres and the risk of cancer is directly related and explains why smokers, whose telomeres shorten at a faster rate because it's a DNA stressor, are more likely to get cancer (26, 32).
Discussion
The primary functions of telomeres is to serve as protective caps for the intrachromosomal genome and prevent any unnecessary DNA repair mechanisms from acting upon the intrachromosomal DNA. Telomeres affect everything around them; the cell cycle, health, productivity, and longevity of the cell, which is why it is imperative that they be fully replicated and have perfect conditions for TERT and TR to assemble with one another as well as bind to the telomere. The end replication problem raises the concern of telomerase function insufficiency and its detrimental impacts on genome integrity. Although there are many theories as to why telomerase becomes less productive throughout an individual's lifespan, the most heavily researched ones are based on the idea that the telomeres are abundant in number but are inhibited from attaching to the telomere and carrying out their function due to external compounds such as shelterin or weak TERT-TR interactions. Furthermore, because the cell is stressed under the circumstance that the telomere is shortening, DNA repair mechanisms and telomere protection-regulation-elongation compounds (TRF1, TRF2, hRap, POT1, and TPP) crowd the telomere and reduce the binding area for the telomerase. Depending on the cell type and the health of the cell, it could either experience an unhealthy shortening of the telomere, mutate the intrachromosomal genome, and enter apoptosis or the cell may try to prevent this crisis by re-activating the TERT proteins and the telomeres will lengthen to an appropriate size, becoming oncogenic in the process. This leads to the question, “if the medicine field were to manipulate telomere length and the regulative proteins surrounding this concept, is it ethically correct?” Without any manipulation of the telomere, we risk the chance of the individual experiencing malignant age-related diseases that will most likely impact them for the rest of their life. Alternatively, as we have seen in cells that have re-activated their TERT proteins in an attempt to prevent crisis, if we were to successfully lengthen the telomere and activate the lengthening proteins, this gives the cell the potential to be cancerous because it is overcoming the Hayflick limit. By giving this cell the ability to divide an innumerable times, we are giving it cancer. The cell can go one of 2 ways, but because each way most likely results in cancer, either there is another way to overcome this issue or there is simply no way to manipulate the genome without it resulting in tumor formation.
Conclusion
Although the solution to cellular immortality has been in plain sight for ages, it has only recently become clear how powerfully transformative it could be for humanity. The only thing standing between humanity and a completely different world essentially comes down to a couple enzymes and some DNA. Telomerase, a Nobel prize winning discovery, is a remarkable enzyme capable of repairing telomeres using only a TERT protein and TR. Conversely, it is equally capable of remaining dormant and inactive for decades upon decades. We still await the answer to the question of how telomerase is activated, but theories have elicited many intriguing lines of thought including external stimuli and interactions between telomerase enzymes and other telomere-based compounds. We do know, however, that the makeup of our telomeres and how they are repaired is what allows us to stay alive but is also the reason that we eventually die. Many problems appear throughout the journey that a telomerase enzyme takes in its path to restore the telomere, and each one raises more and more questions; all of which suggest that the FDA may eventually approve telomerase clinical trials to increase our understanding of these extraordinary enzymes. The future for telomerase studies is bright, but much research must be done if we want to fill the gaps in the outstanding process we know as telomerase activity and telomere elongation.
Acknowledgements
The author would like to thank Drs. Hazal Gezmis and Hakan Coskun for their constant guidance and assistance throughout the entirety of this review.
References
Aksenova, Anna Y., and Sergei M. Mirkin. “At the Beginning of the End and in the Middle of the Beginning: Structure and Maintenance of Telomeric DNA Repeats and Interstitial Telomeric Sequences.” Genes, vol. 10, no. 2, Feb. 2019, p. 118. PubMed Central, https://doi.org/10.3390/genes10020118 (2)
Hayflick, L. “THE LIMITED IN VITRO LIFETIME OF HUMAN DIPLOID CELL STRAINS.” Experimental Cell Research, vol. 37, Mar. 1965, pp. 614–36. PubMed, https://doi.org/10.1016/0014-4827(65)90211-9 (13)
Chow, Tracy T., et al. “Early and Late Steps in Telomere Overhang Processing in Normal Human Cells: The Position of the Final RNA Primer Drives Telomere Shortening.” Genes & Development, vol. 26, no. 11, June 2012, pp. 1167–78. PubMed, https://doi.org/10.1101/gad.187211.112 (4)
Dokudovskaya, S. S., et al. “Telomerase Is an Unusual RNA-Containing Enzyme. A Review.” Biochemistry. Biokhimiia, vol. 62, no. 11, Nov. 1997, pp. 1206–15 (10)
Farkash, Evan A., and Eline T. Luning Prak. “DNA Damage and L1 Retrotransposition.” Journal of Biomedicine and Biotechnology, vol. 2006, 2006, p. 37285. PubMed Central, https://doi.org/10.1155/JBB/2006/37285. (11)
TERF1 Telomeric Repeat Binding Factor 1 [Homo Sapiens (Human)] - Gene - NCBI. https://www.ncbi.nlm.nih.gov/gene/7013#:~:text=TRF1%20links%20TopoIIalpha%20and%20SAC,with%20TRF1%20in%20cancer%20cells. Accessed 9 Oct. 2023 (29)
Maestroni, Laetitia, et al. “Solving the Telomere Replication Problem.” Genes, vol. 8, no. 2, Jan. 2017, p. 55. PubMed Central, https://doi.org/10.3390/genes8020055 (18)
Zakian, Virginia A. “Telomeres: The Beginnings and Ends of Eukaryotic Chromosomes.” Experimental Cell Research, vol. 318, no. 12, July 2012, pp. 1456–60. PubMed Central, https://doi.org/10.1016/j.yexcr.2012.02.015 (33)
Shammas, Masood A. “Telomeres, Lifestyle, Cancer, and Aging.” Current Opinion in Clinical Nutrition and Metabolic Care, vol. 14, no. 1, Jan. 2011, pp. 28–34. PubMed Central, https://doi.org/10.1097/MCO.0b013e32834121b1 (26)
Yao, Yixin, and Wei Dai. “Genomic Instability and Cancer.” Journal of Carcinogenesis & Mutagenesis, vol. 5, 2014, p. 1000165. PubMed Central, https://doi.org/10.4172/2157-2518.1000165 (32)
Mason, Philip J., and Nieves Perdigones. “Telomere Biology and Translational Research.” Translational Research : The Journal of Laboratory and Clinical Medicine, vol. 162, no. 6, Dec. 2013, pp. 333–42. PubMed Central, https://doi.org/10.1016/j.trsl.2013.08.009 (19)
Corey, David R. “Telomeres and Telomerase: From Discovery to Clinical Trials.” Chemistry & Biology, vol. 16, no. 12, Dec. 2009, pp. 1219–23. PubMed Central, https://doi.org/10.1016/j.chembiol.2009.12.001 (7)
Podlevsky, Joshua D., and Julian J. L. Chen. “Evolutionary Perspectives of Telomerase RNA Structure and Function.” RNA Biology, vol. 13, no. 8, June 2016, pp. 720–32. PubMed Central, https://doi.org/10.1080/15476286.2016.1205768 (24)
Dey, Abhishek, and Kausik Chakrabarti. “Current Perspectives of Telomerase Structure and Function in Eukaryotes with Emerging Views on Telomerase in Human Parasites.” International Journal of Molecular Sciences, vol. 19, no. 2, Jan. 2018, p. 333. PubMed Central, https://doi.org/10.3390/ijms19020333 (9)
Zvereva, M. I., et al. “Telomerase: Structure, Functions, and Activity Regulation.” Biochemistry. Biokhimiia, vol. 75, no. 13, Dec. 2010, pp. 1563–83. PubMed, https://doi.org/10.1134/s0006297910130055 (34)
Rahnama, Mostafa, et al. “Telomere Roles in Fungal Genome Evolution and Adaptation.” Frontiers in Genetics, vol. 12, Aug. 2021, p. 676751. PubMed Central, https://doi.org/10.3389/fgene.2021.676751 (25)
Telomeres. https://www.mun.ca/biology/scarr/Telomeres.html. Accessed 14 Nov. 2023. (28)
Jády, Beáta E., et al. “Cell Cycle-Dependent Recruitment of Telomerase RNA and Cajal Bodies to Human Telomeres.” Molecular Biology of the Cell, vol. 17, no. 2, Feb. 2006, pp. 944–54. PubMed Central, https://doi.org/10.1091/mbc.E05-09-0904 (15)
Matulić, M., et al. “Telomere Dynamics: The Means to an End.” Cell Proliferation, vol. 40, no. 4, July 2007, pp. 462–74. PubMed Central, https://doi.org/10.1111/j.1365-2184.2007.00452.x (20)
Siderakis, Manos, and Madalena Tarsounas. “Telomere Regulation and Function during Meiosis.” Chromosome Research : An International Journal on the Molecular, Supramolecular and Evolutionary Aspects of Chromosome Biology, vol. 15, no. 5, 2007, pp. 667–79. PubMed Central, https://doi.org/10.1007/s10577-007-1149-7 (27)
O’Sullivan, Roderick J., and Jan Karlseder. “Telomeres: Protecting Chromosomes against Genome Instability.” Nature Reviews. Molecular Cell Biology, vol. 11, no. 3, Mar. 2010, pp. 171–81. PubMed Central, https://doi.org/10.1038/nrm2848 (23)
Collins, Kathleen. “The Biogenesis and Regulation of Telomerase Holoenzymes.” Nature Reviews. Molecular Cell Biology, vol. 7, no. 7, July 2006, pp. 484–94. PubMed Central, https://doi.org/10.1038/nrm1961 (6)
Collins, Kathleen. “Single-Stranded DNA Repeat Synthesis by Telomerase.” Current Opinion in Chemical Biology, vol. 15, no. 5, Oct. 2011, pp. 643–48. PubMed Central, https://doi.org/10.1016/j.cbpa.2011.07.011 (5)
Fulnečková, Jana, et al. “Telomerase Interaction Partners–Insight from Plants.” International Journal of Molecular Sciences, vol. 23, no. 1, Dec. 2021, p. 368. PubMed Central, https://doi.org/10.3390/ijms23010368. (12)
Mir, Seyed Mostafa, et al. “Shelterin Complex at Telomeres: Implications in Ageing.” Clinical Interventions in Aging, vol. 15, June 2020, pp. 827–39. PubMed Central, https://doi.org/10.2147/CIA.S256425 (21)
Muraki, Keiko, et al. “Mechanisms of Telomere Loss and Their Consequences for Chromosome Instability.” Frontiers in Oncology, vol. 2, Oct. 2012, p. 135. PubMed Central, https://doi.org/10.3389/fonc.2012.00135 (22)
Adwan Shekhidem, Huda, et al. “Telomeres and Longevity: A Cause or an Effect?” International Journal of Molecular Sciences, vol. 20, no. 13, July 2019, p. 3233. PubMed Central, https://doi.org/10.3390/ijms20133233 (1)
TERT Telomerase Reverse Transcriptase [Homo Sapiens (Human)] - Gene - NCBI. https://www.ncbi.nlm.nih.gov/gene/7015. Accessed 9 Oct. 2023 (30)
Chakravarti, Deepavali, et al. “Telomeres: History, Health and Hallmarks of Aging.” Cell, vol. 184, no. 2, Jan. 2021, pp. 306–22. PubMed Central, https://doi.org/10.1016/j.cell.2020.12.028 (3)
Heacock, Michelle, et al. “Molecular Analysis of Telomere Fusions in Arabidopsis: Multiple Pathways for Chromosome End-Joining.” The EMBO Journal, vol. 23, no. 11, June 2004, pp. 2304–13. PubMed Central, https://doi.org/10.1038/sj.emboj.7600236 (14)
Lowden, Mia Rochelle, et al. “DNA Synthesis Generates Terminal Duplications That Seal End-to-End Chromosome Fusions.” Science (New York, N.Y.), vol. 332, no. 6028, Apr. 2011, pp. 468–71. PubMed Central, https://doi.org/10.1126/science.1199022 (17)
Lee, Jenna, and Mark V. Pellegrini. “Biochemistry, Telomere And Telomerase.” StatPearls, StatPearls Publishing, 2023. PubMed, http://www.ncbi.nlm.nih.gov/books/NBK576429/ (16)
de Jesus, Bruno Bernardes, and Maria A. Blasco. “Telomerase at the Intersection of Cancer and Aging.” Trends in Genetics : TIG, vol. 29, no. 9, Sept. 2013, pp. 513–20. PubMed Central, https://doi.org/10.1016/j.tig.2013.06.007 (8)
Tsoukalas, Dimitris, et al. “Discovery of Potent Telomerase Activators: Unfolding New Therapeutic and Anti-Aging Perspectives.” Molecular Medicine Reports, vol. 20, no. 4, Oct. 2019, pp. 3701–08. PubMed Central, https://doi.org/10.3892/mmr.2019.10614 (31)
Abbreviations
DNA- Deoxyribonucleic acid
RNA- Ribonucleic acid
TERT- Telomerase reverse transcriptase
RNP- Ribonucleoprotein
RT- Reverse transcriptase
RDR- Recombination- dependent replication
TR- Telomere RNA
TRF1- Telomere repeat binding factor 1
ATP- Adenosine triphosphate
Kommentare